Towards the directed evolution of protein materials
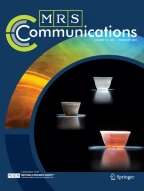
Protein-based materials are a powerful instrument for a new generation of biological materials, with many chemical and mechanical capabilities. Through the manipulation of DNA, researchers can design proteins at the molecular level, engineering a vast array of structural building blocks. However, our capability to rationally design and predict the properties of such materials is limited by the vastness of possible sequence space. Directed evolution has emerged as a powerful tool to improve biological systems through mutation and selection, presenting another avenue to produce novel protein materials. In this prospective review, we discuss the application of directed evolution for protein materials, reviewing current examples and developments that could facilitate the evolution of protein for material applications.
This is a preview of subscription content, log in via an institution to check access.
Access this article
Subscribe and save
Springer+ Basic
€32.70 /Month
- Get 10 units per month
- Download Article/Chapter or eBook
- 1 Unit = 1 Article or 1 Chapter
- Cancel anytime
Buy Now
Price includes VAT (France)
Instant access to the full article PDF.
Rent this article via DeepDyve
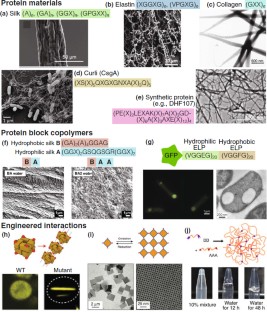
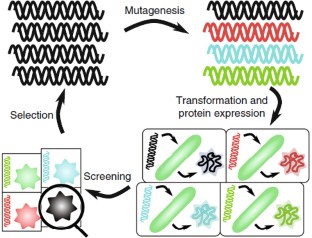
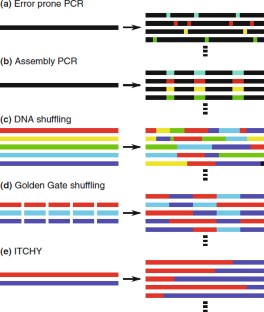
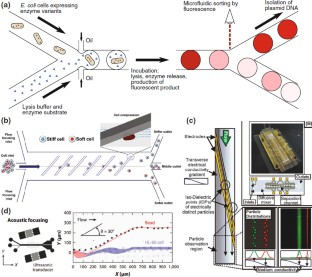
Similar content being viewed by others
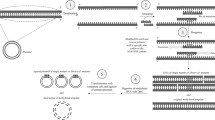
Tailoring Proteins to Re-Evolve Nature: A Short Review
Article 27 September 2018
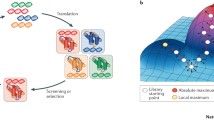
Methods for the directed evolution of proteins
Article 09 June 2015
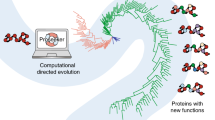
In silico evolution of nucleic acid-binding proteins from a nonfunctional scaffold
Article 24 February 2022
Explore related subjects
References
- A.A. Cheng and T.K. Lu: Synthetic biology: an emerging engineering discipline. Annu. Rev. Biomed. Eng.14, 155–178 (2012). ArticleCASGoogle Scholar
- Y.-J. Chen. P. Liu, A.A.K. Nielsen, J.A.N. Brophy, K. Clancy, T. Peterson, and C.A. Voigt: Characterization of 582 natural and synthetic terminators and quantification of their design constraints. Nat. Methods10, 659–664 (2013). ArticleCASGoogle Scholar
- A.A.K. Nielsen, B.S. Der, J. Shin, P. Vaidyanathan, V. Paralanov, E.A. Strychalski, D. Ross, D. Densmore, and C.A. Voigt: Genetic circuit design automation. Science352, aac7341 (2016). ArticleGoogle Scholar
- K.M. Esvelt and H.H. Wang: Genome-scale engineering for systems and synthetic biology. Mol. Syst. Biol.9, 641 (2013). ArticleGoogle Scholar
- M.T. Mee, J.J. Collins, G.M. Church, and H.H. Wang: Syntrophic exchange in synthetic microbial communities. Proc. Natl. Acad. Sci. USA111, E2149–E2156 (2014). ArticleCASGoogle Scholar
- M. Elowitz and W.A. Lim: Build life to understand it. Nature468, 889–890 (2010). ArticleCASGoogle Scholar
- C.J. Paddon, P.J. Westfall, D.J. Pitera, K. Benjamin, K. Fisher, D. McPhee, M.D. Leavell, A. Tai, A. Main, D. Eng, D.R. Polichuk, K.H. Teoh, D.W. Reed, T. Treynor, J. Lenihan, H. Jiang, M. Fleck, S. Bajad, G. Dang, D. Dengrove, D. Diola, G. Dorin, K.W. Ellens, S. Fickes, J. Galazzo, S.P. Gaucher, T. Geistlinger, R. Henry, M. Hepp, T. Horning, T. Iqbal, L. Kizer, B. Lieu, D. Melis, N. Moss, R. Regentin, S. Secrest, H. Tsuruta, R. Vazquez, L.F. Westblade, L. Xu, M. Yu, Y. Zhang, L. Zhao, J. Lievense, P.S. Covello, J.D. Keasling, K.K. Reiling, N.S. Renninger, and J.D. Newman: High-level semi-synthetic production of the potent antimalarial artemisinin. Nature496, 528–532 (2013). ArticleCASGoogle Scholar
- P. Ball: Synthetic biology—Engineering nature to make materials. MRS Bull.43, 477–484 (2018). ArticleGoogle Scholar
- R.A. Le Feuvre and N.S. Scrutton: A living foundry for synthetic biological materials: a synthetic biology roadmap to new advanced materials. Synth. Syst. Biotechnol.3, 105–112 (2018). ArticleGoogle Scholar
- M.K. Rice and W.C. Ruder: Creating biological nanomaterials using synthetic biology. Sci. Technol. Adv. Mater.15, 014401 (2013). ArticleGoogle Scholar
- S.R. MacEwan and A. Chilkoti: Applications of elastin-like polypeptides in drug delivery. J. Controlled Release190, 314–330 (2014). ArticleCASGoogle Scholar
- K.G. DeFrates, R. Moore, J. Borgesi, G. Lin, T. Mulderig, V. Beachley, and X. Hu: Protein-based fiber materials in medicine: a review. Nanomaterials (Basel, Switz.)8, 457 (2018). ArticleGoogle Scholar
- N.H. Romano, D. Sengupta, C. Chung, and S.C. Heilshorn: Protein-engineered biomaterials: nanoscale mimics of the extracellular matrix. Biochim. Biophys. Acta1810, 339–349 (2011). ArticleCASGoogle Scholar
- G. Chan and D.J. Mooney: New materials for tissue engineering: towards greater control over the biological response. Trends Biotechnol.26, 382–392 (2008). ArticleCASGoogle Scholar
- J.M. Caves, V.A. Kumar, A.W. Martinez, J. Kim, C.M. Ripberger, C.A. Haller, and E.L. Chaikof: The use of microfiber composites of elastin-like protein matrix reinforced with synthetic collagen in the design of vascular grafts. Biomaterials31, 7175–7182 (2010). ArticleCASGoogle Scholar
- C. Gilbert and T. Ellis: Biological engineered living materials: growing functional materials with genetically programmable properties. ACS Synth. Biol.8, 1–15 (2019). ArticleCASGoogle Scholar
- P.Q. Nguyen, N.-M.D. Courchesne, A. Duraj-Thatte, P. Praveschotinunt, and N.S. Joshi: Engineered living materials: prospects and challenges for using biological systems to direct the assembly of smart materials. Adv. Mater.30, 1704847 (2018). ArticleGoogle Scholar
- S.E. Naleway, M.M. Porter, J. McKittrick, and M.A. Meyers: Structural design elements in biological materials: application to bioinspiration. Adv. Mater.27, 5455–5476 (2015). ArticleCASGoogle Scholar
- K.A. Dill and J.L. MacCallum: The protein-folding problem, 50 years on. Science338, 1042–1046 (2012). ArticleCASGoogle Scholar
- R.E. Cobb, N. Sun, and H. Zhao: Directed evolution as a powerful synthetic biology tool. Methods60, 81–90 (2013). ArticleCASGoogle Scholar
- J.C.M. van Hest and D.A. Tirrell: Protein-based materials, toward a new level of structural control. Chem. Commun. 1897–1904 (2001). Google Scholar
- Y.J. Yang, A.L. Holmberg, and B.D. Olsen: Artificially engineered protein polymers. Annu. Rev. Chem. Biomol. Eng.8, 549–575 (2017). ArticleCASGoogle Scholar
- L. Yang, A. Liu, S. Cao, R.M. Putri, P. Jonkheijm, and J.J.L.M. Cornelissen: Self-assembly of proteins: towards supramolecular materials. Chem.–Eur. J.22, 15570–15582 (2016). ArticleCASGoogle Scholar
- M.S. Ekiz, G. Cinar, M.A. Khalily, and M.O. Guler: Self-assembled peptide nanostructures for functional materials. Nanotechnology27, 402002 (2016). ArticleGoogle Scholar
- B.O. Okesola and A. Mata: Multicomponent self-assembly as a tool to harness new properties from peptides and proteins in material design. Chem. Soc. Rev.47, 3721–3736 (2018). ArticleCASGoogle Scholar
- C. Vepari and D.L. Kaplan: Silk as a biomaterial. Prog. Polym. Sci.32, 991–1007 (2007). ArticleCASGoogle Scholar
- O. Tokareva, V.A. Michalczechen-Lacerda, E.L. Rech, and D.L. Kaplan: Recombinant DNA production of spider silk proteins. Microb. Biotechnol.6, 651–663 (2013). ArticleCASGoogle Scholar
- Q. Peng, Y. Zhang, L. Lu, H. Shao, K. Qin, X. Hu, and X. Xia: Recombinant spider silk from aqueous solutions via a bio-inspired microfluidic chip. Sci. Rep.6, 36473 (2016). ArticleCASGoogle Scholar
- C.L. Craig: Evolution of arthropod silks. Annu. Rev. Entomol.42, 231–267 (1997). ArticleCASGoogle Scholar
- F. Vollrath and P. Selden: The role of behavior in the evolution of spiders, silks, and webs. Annu. Rev. Ecol. Evol. Syst.38, 819–846 (2007). ArticleGoogle Scholar
- X. Hu, K. Vasanthavada, K. Kohler, S. McNary, A.M.F. Moore, and C.A. Vierra: Molecular mechanisms of spider silk. Cell. Mol. Life Sci.63, 1986–1999 (2006). CASGoogle Scholar
- L. Römer and T. Scheibel: The elaborate structure of spider silk. Prion2, 154–161 (2008). ArticleGoogle Scholar
- L.P. Gage and R.F. Manning: Internal structure of the silk fibroin gene of Bombyx mori. I. The fibroin gene consists of a homogeneous alternating array of repetitious crystalline and amorphous coding sequences. J. Biol. Chem.255, 9444–9450 (1980). ArticleCASGoogle Scholar
- K.M. Rudall and W. Kenchington: Arthropod silks: the problem of fibrous proteins in animal tissues. Annu. Rev. Entomol.16, 73–96 (1971). ArticleCASGoogle Scholar
- A. Rising, H. Nimmervoll, S. Grip, A. Fernandez-Arias, E. Storckenfeldt, D.P. Knight, F. Vollrath, and W. Engström: Spider silk proteins— mechanical property and gene sequence. Zool. Sci.22, 273–281 (2005). ArticleCASGoogle Scholar
- T. Kowalczyk, K. Hnatuszko-Konka, A. Gerszberg, and A.K. Kononowicz: Elastin-like polypeptides as a promising family of genetically-engineered protein based polymers. World J. Microbiol. Biotechnol.30, 2141–2152 (2014). ArticleCASGoogle Scholar
- W. Hassouneh, T. Christensen, and A. Chilkoti: Elastin-like Polypeptides as a Purification Tag for Recombinant Proteins. Curr. Protoc. Protein Sci. Editor. Board John E Coligan Al CHAPTER, Unit–6.11 (2010). Google Scholar
- R. Saxena and M.J. Nanjan: Elastin-like polypeptides and their applications in anticancer drug delivery systems: a review. Drug Deliv.22, 156–167 (2015). ArticleCASGoogle Scholar
- K.E. Inostroza-Brito, E. Collin, O. Siton-Mendelson, K.H. Smith, A. Monge-Marcet, D.S. Ferreira, R.P. Rodríguez, M. Alonso, J.C. Rodríguez-Cabello, R.L. Reis, F. Sagués, L. Botto, R. Bitton, H.S. Azevedo, and A. Mata: Co-assembly, spatiotemporal control and morphogenesis of a hybrid protein–peptide system. Nat. Chem.7, 897 (2015). ArticleCASGoogle Scholar
- H. Wang, A. Paul, D. Nguyen, A. Enejder, and S.C. Heilshorn: Tunable control of hydrogel microstructure by kinetic competition between selfassembly and crosslinking of elastin-like proteins. ACS Appl. Mater. Interfaces10, 21808–21815 (2018). ArticleCASGoogle Scholar
- F.G. Quiroz and A. Chilkoti: Sequence heuristics to encode phase behaviour in intrinsically disordered protein polymers. Nat. Mater.14, 1164 (2015). ArticleCASGoogle Scholar
- N.K. Li, S. Roberts, F.G. Quiroz, A. Chilkoti, and Y.G. Yingling: Sequence directionality dramatically affects LCST behavior of elastin-like polypeptides. Biomacromolecules19, 2496–2505 (2018). ArticleCASGoogle Scholar
- M.D. Shoulders and R.T. Raines: Collagen structure and stability. Annu. Rev. Biochem.78, 929–958 (2009). ArticleCASGoogle Scholar
- K. Gelse, E. Pöschl, and T. Aigner: Collagens—structure, function, and biosynthesis. Adv. Drug Deliv. Rev.55, 1531–1546 (2003). ArticleCASGoogle Scholar
- A.V. Persikov, J.A.M. Ramshaw, and B. Brodsky: Prediction of collagen stability from amino acid sequence. J. Biol. Chem.280, 19343–19349 (2005). ArticleCASGoogle Scholar
- S. Lukomski, K. Nakashima, I. Abdi, V.J. Cipriano, R.M. Ireland, S.D. Reid, G.G. Adams, and J.M. Musser: Identification and characterization of the scl gene encoding a group a streptococcus extracellular protein virulence factor with similarity to human collagen. Infect. Immun.68, 6542–6553 (2000). ArticleCASGoogle Scholar
- Z. Yu, B. An, J.A.M. Ramshaw, and B. Brodsky: Bacterial collagen-like proteins that form triple-helical structures. J. Struct. Biol.186, 451–461 (2014). ArticleCASGoogle Scholar
- E. Cosgriff-Hernandez, M.S. Hahn, B. Russell, T. Wilems, D. Munoz-Pinto, M.B. Browning, J. Rivera, and M. Höök: Bioactive hydrogels based on designer collagens. Acta Biomater.6, 3969–3977 (2010). ArticleCASGoogle Scholar
- M.M. Barnhart and M.R. Chapman: Curli biogenesis and function. Annu. Rev. Microbiol.60, 131–147 (2006). ArticleCASGoogle Scholar
- S.K. Collinson, L. Emödy, K.H. Müller, T.J. Trust, and W.W. Kay: Purification and characterization of thin, aggregative fimbriae from Salmonella enteritidis. J. Bacteriol.173, 4773–4781 (1991). ArticleCASGoogle Scholar
- P.Q. Nguyen, Z. Botyanszki, P.K.R. Tay, and N.S. Joshi: Programmable biofilm-based materials from engineered curli nanofibres. Nat. Commun.5, 4945 (2014). ArticleCASGoogle Scholar
- N.-M. Dorval Courchesne, A. Duraj-Thatte, P.K.R. Tay, P.Q. Nguyen, and N.S. Joshi: Scalable production of genetically engineered nanofibrous macroscopic materials via Filtration. ACS Biomater. Sci. Eng.3, 733–741 (2017). ArticleGoogle Scholar
- P.K.R. Tay, P.Q. Nguyen, and N.S. Joshi: A synthetic circuit for mercury bioremediation using self-assembling functional amyloids. ACS Synth. Biol.6, 1841 (2017). ArticleGoogle Scholar
- A.M. Duraj-Thatte, P. Praveschotinunt, T.R. Nash, F.R. Ward, and N.S. Joshi: Modulating bacterial and gut mucosal interactions with engineered biofilm matrix proteins. Sci. Rep.8, 3475 (2018). ArticleGoogle Scholar
- G. Zeng, B.S. Vad, M.S. Dueholm, G. Christiansen, M. Nilsson, T. Tolker-Nielsen, P.H. Nielsen, R.L. Meyer, and D.E. Otzen: Functional bacterial amyloid increases Pseudomonas biofilm hydrophobicity and stiffness. Front. Microbiol.6, 1099 (2015). ArticleGoogle Scholar
- E. Axpe, A. Duraj-Thatte, Y. Chang, D.-M. Kaimaki, A. Sanchez-Sanchez, H.B. Caliskan, N.-M. Dorval Courchesne, and N.S. Joshi: Fabrication of amyloid curli fibers–alginate nanocomposite hydrogels with enhanced stiffness. ACS Biomater. Sci. Eng.4, 2100–2105 (2018). ArticleCASGoogle Scholar
- M.S. Dueholm, M. Albertsen, D. Otzen, and P.H. Nielsen: Curli functional amyloid systems are phylogenetically widespread and display large diversity in operon and protein structure. PLoS ONE7, e51274 (2012). ArticleCASGoogle Scholar
- N.P. King, J.B. Bale, W. Sheffler, D.E. McNamara, S. Gonen, T. Gonen, T. O. Yeates, and D. Baker: Accurate design of co-assembling multicomponent protein nanomaterials. Nature510, 103–108 (2014). ArticleCASGoogle Scholar
- A. Leaver-Fay, M. Tyka, S.M. Lewis, O.F. Lange, J. Thompson, R. Jacak, K. Kaufman, P.D. Renfrew, C.A. Smith, W. Sheffler, I.W. Davis, S. Cooper, A. Treuille, D.J. Mandell, F. Richter, Y.-E. A. Ban, S.J. Fleishman, J.E. Corn, D.E. Kim, S. Lyskov, M. Berrondo, S. Mentzer, Z. Popović, J.J. Havranek, J. Karanicolas, R. Das, J. Meiler, T. Kortemme, J.J. Gray, B. Kuhlman, D. Baker, and P. Bradley: Rosetta3: an object-oriented software suite for the simulation and design of macromolecules. Methods Enzymol.487, 545–574 (2011). ArticleCASGoogle Scholar
- H. Shen, J.A. Fallas, E. Lynch, W. Sheffler, B. Parry, N. Jannetty, J. Decarreau, M. Wagenbach, J.J. Vicente, J. Chen, L. Wang, Q. Dowling, G. Oberdorfer, L. Stewart, L. Wordeman, J.D. Yoreo, C. Jacobs-Wagner, J. Kollman, and D. Baker: De novo design of selfassembling helical protein filaments. Science362, 705–709 (2018). ArticleCASGoogle Scholar
- A.S. Cristie-David, A. Sciore, S. Badieyan, J.D. Escheweiler, P. Koldewey, J.C.A. Bardwell, B.T. Ruotolo, and E.N.G. Marsh: Evaluation of de novodesigned coiled coils as off-the-shelf components for protein assembly. Mol. Syst. Des. Eng.2, 140–148 (2017). ArticleCASGoogle Scholar
- J.M. Fletcher, R.L. Harniman, F.R.H. Barnes, A.L. Boyle, A. Collins, J. Mantell, T.H. Sharp, M. Antognozzi, P.J. Booth, N. Linden, M.J. Miles, R.B. Sessions, P. Verkade, and D.N. Woolfson: Self-assembling cages from coiled-coil peptide modules. Science340, 595–599 (2013). ArticleCASGoogle Scholar
- F. Thomas, W.M. Dawson, E.J.M. Lang, A.J. Burton, G.J. Bartlett, G.G. Rhys, A.J. Mulholland, and D.N. Woolfson: De novo-designed α-helical barrels as receptors for small molecules. ACS Synth. Biol.7, 1808 (2018). ArticleCASGoogle Scholar
- A. Ljubetič, F. Lapenta, H. Gradišar, I. Drobnak, J. Aupič, Ž. Strmšek, D. Lainšček, I. Hafner-Bratkovič, A. Majerle, N. Krivec, M. Benčina, T. Pisanski, T. Ć. Veličković, A. Round, J.M. Carazo, R. Melero, and R. Jerala: Design of coiled-coil protein-origami cages that self-assemble in vitro and in vivo. Nat. Biotechnol.35, 1094 (2017). ArticleGoogle Scholar
- O.S. Rabotyagova, P. Cebe, and D.L. Kaplan: Protein-based block copolymers. Biomacromolecules12, 269–289 (2011). ArticleCASGoogle Scholar
- R. Valluzzi, S. Winkler, D. Wilson, and D.L. Kaplan: Silk: molecular organization and control of assembly. Philos. Trans. R. Soc. B: Biol. Sci.357, 165–167 (2002). ArticleCASGoogle Scholar
- O.S. Rabotyagova, P. Cebe, and D.L. Kaplan: Self-assembly of genetically engineered spider silk block copolymers. Biomacromolecules10, 229–236 (2009). ArticleCASGoogle Scholar
- M.C. Huber, A. Schreiber, P. von Olshausen, B.R. Varga, O. Kretz, B. Joch, S. Barnert, R. Schubert, S. Eimer, P. Kele, and S.M. Schiller: Designer amphiphilic proteins as building blocks for the intracellular formation of organelle-like compartments. Nat. Mater.14, 125 (2015). ArticleCASGoogle Scholar
- N. Dinjaski and D.L. Kaplan: Recombinant protein blends: silk beyond natural design. Curr. Opin. Biotechnol.39, 1–7 (2016). ArticleCASGoogle Scholar
- A.Y. Chen, Z. Deng, A.N. Billings, U.O.S. Seker, M.Y. Lu, R.J. Citorik, B. Zakeri, and T.K. Lu: Synthesis and patterning of tunable multiscale materials with engineered cells. Nat. Mater.13, 515–523 (2014). ArticleCASGoogle Scholar
- J.K. Polka, S.G. Hays, and P.A. Silver: Building spatial synthetic biology with compartments, scaffolds, and communities. Cold Spring Harb. Perspect. Biol.8, a024018 (2016). ArticleGoogle Scholar
- H. Garcia-Seisdedos, C. Empereur-Mot, N. Elad, and E.D. Levy: Proteins evolve on the edge of supramolecular self-assembly. Nature548, 244 (2017). ArticleCASGoogle Scholar
- Y. Suzuki, G. Cardone, D. Restrepo, P.D. Zavattieri, T.S. Baker, and F.A. Tezcan: Self-assembly of coherently dynamic, auxetic, two-dimensional protein crystals. Nature533, 369–373 (2016). ArticleCASGoogle Scholar
- B. Zakeri, J.O. Fierer, E. Celik, E.C. Chittock, U. Schwarz-Linek, V.T. Moy, and M. Howarth: Peptide tag forming a rapid covalent bond to a protein, through engineering a bacterial adhesin. Proc. Natl. Acad. Sci. USA109, E690–E697 (2012). ArticleCASGoogle Scholar
- F. Sun, W.-B. Zhang, A. Mahdavi, F.H. Arnold, and D.A. Tirrell: Synthesis of bioactive protein hydrogels by genetically encoded SpyTag-SpyCatcher chemistry. Proc. Natl. Acad. Sci. USA111, 11269–11274 (2014). ArticleCASGoogle Scholar
- R.E. Cobb, R. Chao and H. Zhao: Directed evolution: past, present and future. AIChE J. Am. Inst. Chem. Eng.59, 1432–1440 (2013). ArticleCASGoogle Scholar
- K.L. Tee and T.S. Wong: Polishing the craft of genetic diversity creation in directed evolution. Biotechnol. Adv.31, 1707–1721 (2013). ArticleCASGoogle Scholar
- M.S. Packer and D.R. Liu: Methods for the directed evolution of proteins. Nat. Rev. Genet.16, 379–394 (2015). ArticleCASGoogle Scholar
- F.H. Arnold: Design by directed evolution. Acc. Chem. Res.31, 125–131 (1998). ArticleCASGoogle Scholar
- A. Currin, N. Swainston, P.J. Day, and D.B. Kell: Synthetic biology for the directed evolution of protein biocatalysts: navigating sequence space intelligently. Chem. Soc. Rev.44, 1172–1239 (2015). ArticleCASGoogle Scholar
- W.P.C. Stemmer: Rapid evolution of a protein in vitro by DNA shuffling. Nature370, 389–391 (1994). ArticleCASGoogle Scholar
- H. Zhao and F.H. Arnold: Optimization of DNA shuffling for high fidelity recombination. Nucleic Acids Res.25, 1307–1308 (1997). ArticleCASGoogle Scholar
- A. Crameri, S.-A. Raillard, E. Bermudez, and W.P.C. Stemmer: DNA shuffling of a family of genes from diverse species accelerates directed evolution. Nature391, 288–291 (1998). ArticleCASGoogle Scholar
- C. Engler, R. Gruetzner, R. Kandzia, and S. Marillonnet: Golden gate shuffling: a one-pot DNA shuffling method based on type IIs restriction enzymes. PLoS ONE4, e5553 (2009). ArticleGoogle Scholar
- M. Ostermeier, J.H. Shim, and S.J. Benkovic: A combinatorial approach to hybrid enzymes independent of DNA homology. Nat. Biotechnol.17, 1205 (1999). ArticleCASGoogle Scholar
- D. Bikard, S. Julié-Galau, G. Cambray, and D. Mazel: The synthetic integron: an in vivo genetic shuffling device. Nucleic Acids Res.38, e153–e153 (2010). ArticleGoogle Scholar
- P.L. Foster: In vivo mutagenesis. Methods Enzymol.204, 114–125 (1991). ArticleCASGoogle Scholar
- H.H. Wang, F.J. Isaacs, P.A. Carr, Z.Z. Sun, G. Xu, C.R. Forest, and G.M. Church: Programming cells by multiplex genome engineering and accelerated evolution. Nature460, 894–898 (2009). ArticleCASGoogle Scholar
- S.O. Halperin, C.J. Tou, E.B. Wong, C. Modavi, D.V. Schaffer, and J.E. Dueber: CRISPR-guided DNA polymerases enable diversification of all nucleotides in a tunable window. Nature560, 248 (2018). ArticleCASGoogle Scholar
- S. Lutz: Beyond directed evolution—semi-rational protein engineering and design. Curr. Opin. Biotechnol.21, 734–743 (2010). ArticleCASGoogle Scholar
- P. Heinzelman, C.D. Snow, I. Wu, C. Nguyen, A. Villalobos, S. Govindarajan, J. Minshull, and F.H. Arnold: A family of thermostable fungal cellulases created by structure-guided recombination. Proc. Natl. Acad. Sci. USA106, 5610–5615 (2009). ArticleCASGoogle Scholar
- P.A.G. Tizei, E. Csibra, L. Torres, and V.B. Pinheiro: Selection platforms for directed evolution in synthetic biology. Biochem. Soc. Trans.44, 1165–1175 (2016). ArticleCASGoogle Scholar
- S. Raman, J.K. Rogers, N.D. Taylor, and G.M. Church: Evolution-guided optimization of biosynthetic pathways. Proc. Natl. Acad. Sci. USA111, 17803–17808 (2014). ArticleCASGoogle Scholar
- J.-D. Pédelacq, S. Cabantous, T. Tran, T.C. Terwilliger, and G.S. Waldo: Engineering and characterization of a superfolder green fluorescent protein. Nat. Biotechnol.24, 79–88 (2006). ArticleGoogle Scholar
- S.-A. Morgan, D.C. Nadler, R. Yokoo, and D.F. Savage: Biofuel metabolic engineering with biosensors. Curr. Opin. Chem. Biol.35, 150–158 (2016). ArticleCASGoogle Scholar
- S.R.A. Devenish, M. Kaltenbach, M. Fischlechner, and F. Hollfelder: Droplets as Reaction Compartments for Protein Nanotechnology. In Protein Nanotechnology: Protocols, Instrumentation, and Applications, 2nd ed., edited by J A. Gerrard (Humana Press, 2013), pp. 269–286. ChapterGoogle Scholar
- K.T. O’Neil and R.H. Hoess: Phage display: protein engineering by directed evolution. Curr. Opin. Struct. Biol.5, 443–449 (1995). ArticleGoogle Scholar
- A. Fernandez-Gacio, M. Uguen, and J. Fastrez: Phage display as a tool for the directed evolution of enzymes. Trends Biotechnol.21, 408–414 (2003). ArticleCASGoogle Scholar
- K.M. Esvelt, J.C. Carlson, and D.R. Liu: A system for the continuous directed evolution of biomolecules. Nature472, 499 (2011). ArticleCASGoogle Scholar
- H. Leemhuis, V. Stein, A.D. Griffiths, and F. Hollfelder: New genotype–phenotype linkages for directed evolution of functional proteins. Curr. Opin. Struct. Biol.15, 472–478 (2005). ArticleCASGoogle Scholar
- S. Kosuri, D.B. Goodman, G. Cambray, V.K. Mutalik, Y. Gao, A.P. Arkin, D. Endy, and G.M. Church: Composability of regulatory sequences controlling transcription and translation in Escherichia coli. Proc. Natl. Acad. Sci. USA.110, 14024 (2013). ArticleCASGoogle Scholar
- A. Zinchenko, S.R.A. Devenish, B. Kintses, P.-Y. Colin, M. Fischlechner, and F. Hollfelder: One in a million: flow cytometric sorting of single celllysate assays in monodisperse picolitre double emulsion droplets for directed evolution. Anal. Chem.86, 2526–2533 (2014). ArticleCASGoogle Scholar
- J.J. Agresti, E. Antipov, A.R. Abate, K. Ahn, A.C. Rowat, J.-C. Baret, M. Marquez, A.M. Klibanov, A.D. Griffiths, and D.A. Weitz: Ultrahigh-throughput screening in drop-based microfluidics for directed evolution. Proc. Natl. Acad. Sci. USA107, 4004–4009 (2010). ArticleCASGoogle Scholar
- P.-Y. Colin, B. Kintses, F. Gielen, C.M. Miton, G. Fischer, M.F. Mohamed, M. Hyvönen, D.P. Morgavi, D.B. Janssen, and F. Hollfelder: Ultrahigh-throughput discovery of promiscuous enzymes by picodroplet functional metagenomics. Nat. Commun.6, 10008 (2015). ArticleCASGoogle Scholar
- F. Gielen, R. Hours, S. Emond, M. Fischlechner, U. Schell, and F. Hollfelder: Ultrahigh-throughput–directed enzyme evolution by absorbance-activated droplet sorting (AADS). Proc. Natl. Acad. Sci. USA113, E7383–E7389 (2016). ArticleCASGoogle Scholar
- M. Girault, H. Kim, H. Arakawa, K. Matsuura, M. Odaka, A. Hattori, H. Terazono, and K. Yasuda: An on-chip imaging droplet-sorting system: a real-time shape recognition method to screen target cells in droplets with single cell resolution. Sci. Rep.7, 40072 (2017). ArticleCASGoogle Scholar
- H.-D. Xi, H. Zheng, W. Guo, A.M. Gañán-Calvo, Y. Ai, C.-W. Tsao, J. Zhou, W. Li, Y. Huang, N.-T. Nguyen, and S.H. Tan: Active droplet sorting in microfluidics: a review. Lab Chip17, 751–771 (2017). ArticleCASGoogle Scholar
- S.S. Terekhov, I.V. Smirnov, A.V. Stepanova, T.V. Bobik, Y.A. Mokrushina, N.A. Ponomarenko, A.A. Belogurov, M.P. Rubtsova, O.V. Kartseva, M.O. Gomzikova, A.A. Moskovtsev, A.S. Bukatin, M.V. Dubina, E.S. Kostryukova, V.V. Babenko, M.T. Vakhitova, A.I. Manolov, M.V. Malakhova, M.A. Kornienko, A.V. Tyakht, A.A. Vanyushkina, E.N. Ilina, P. Masson, A.G. Gabibov, and S. Altman: Microfluidic droplet platform for ultrahigh-throughput single-cell screening of biodiversity. Proc. Natl. Acad. Sci. USA114, 2550–2555 (2017). ArticleCASGoogle Scholar
- P.A. Romero and F.H. Arnold: Exploring protein fitness landscapes by directed evolution. Nat. Rev. Mol. Cell Biol.10, 866–876 (2009). ArticleCASGoogle Scholar
- J.D. Bloom and F.H. Arnold: In the light of directed evolution: pathways of adaptive protein evolution. Proc. Natl. Acad. Sci. USA106, 9995–10000 (2009). ArticleCASGoogle Scholar
- S. Kauffman and S. Levin: Towards a general theory of adaptive walks on rugged landscapes. J. Theor. Biol.128, 11–45 (1987). ArticleCASGoogle Scholar
- J.G. Heddle, S. Chakraborti, and K. Iwasaki: Natural and artificial protein cages: design, structure and therapeutic applications. Curr. Opin. Struct. Biol.43, 148–155 (2017). ArticleCASGoogle Scholar
- B. Wörsdörfer, K.J. Woycechowsky, and D. Hilvert: Directed evolution of a protein container. Science331, 589–592 (2011). ArticleGoogle Scholar
- G.L. Butterfield, M.J. Lajoie, H.H. Gustafson, D.L. Sellers, U. Nattermann, D. Ellis, J.B. Bale, S. Ke, G.H. Lenz, A. Yehdego, R. Ravichandran, S.H. Pun, N.P. King, and D. Baker: Evolution of a designed protein assembly encapsulating its own RNA genome. Nature552, 415–420 (2017). ArticleCASGoogle Scholar
- J.B. Bale, S. Gonen, Y. Liu, W. Sheffler, D. Ellis, C. Thomas, D. Cascio, T. O. Yeates, T. Gonen, N.P. King, and D. Baker: Accurate design of megadalton-scale two-component icosahedral protein complexes. Science353, 389–394 (2016). ArticleCASGoogle Scholar
- N.C. Tang and A. Chilkoti: Combinatorial codon scrambling enables scalable gene synthesis and amplification of repetitive proteins. Nat. Mater.15, 419 (2016). ArticleCASGoogle Scholar
- N.G. Bednarska, J. Schymkowitz, F. Rousseau, and J. Van Eldere: Protein aggregation in bacteria: the thin boundary between functionality and toxicity. Microbiology159, 1795–1806 (2013). ArticleCASGoogle Scholar
- M.L. Evans, E. Chorell, J.D. Taylor, J. Åden, A. Götheson, F. Li, M. Koch, L. Sefer, S.J. Matthews, P. Wittung-Stafshede, F. Almqvist, and M.R. Chapman: The bacterial curli system possesses a potent and selective inhibitor of amyloid formation. Mol. Cell57, 445–455 (2015). ArticleCASGoogle Scholar
- B. Kintses, C. Hein, M.F. Mohamed, M. Fischlechner, F. Courtois, C. Lainé, and F. Hollfelder: Picoliter cell lysate assays in microfluidic droplet compartments for directed enzyme evolution. Chem. Biol.19, 1001–1009 (2012). ArticleCASGoogle Scholar
- Cappello Joseph, Crissman John, Dorman Mary, Mikolajczak Marcia, Textor Garret, Marquet Magda, and Ferrari Franco: Genetic engineering of structural protein polymers. Biotechnol. Prog.6, 198–202 (1990). ArticleGoogle Scholar
- M.C. Huber, A. Schreiber, W. Wild, K. Benz, and S.M. Schiller: Introducing a combinatorial DNA-toolbox platform constituting defined protein-based biohybrid-materials. Biomaterials35, 8767–8779 (2014). ArticleCASGoogle Scholar
- E.M. Darling and D. Di Carlo: High-throughput assessment of cellular mechanical properties. Annu. Rev. Biomed. Eng.17, 35–62 (2015). ArticleCASGoogle Scholar
- N. Nitta, T. Sugimura, A. Isozaki, H. Mikami, K. Hiraki, S. Sakuma, T. Iino, F. Arai, T. Endo, Y. Fujiwaki, H. Fukuzawa, M. Hase, T. Hayakawa, K. Hiramatsu, Y. Hoshino, M. Inaba, T. Ito, H. Karakawa, Y. Kasai, K. Koizumi, S. Lee, C. Lei, M. Li, T. Maeno, S. Matsusaka, D. Murakami, A. Nakagawa, Y. Oguchi, M. Oikawa, T. Ota, K. Shiba, H. Shintaku, Y. Shirasaki, K. Suga, Y. Suzuki, N. Suzuki, Y. Tanaka, H. Tezuka, C. Toyokawa, Y. Yalikun, M. Yamada, M. Yamagishi, T. Yamano, A. Yasumoto, Y. Yatomi, M. Yazawa, D. Di Carlo, Y. Hosokawa, S. Uemura, Y. Ozeki, and K. Goda: Intelligent image-activated cell sorting. Cell, 175, 266–276.e13 (2018). ArticleCASGoogle Scholar
- J.S. Dudani, D.R. Gossett, H.T.K. Tse, and D.D. Carlo: Pinched-flow hydrodynamic stretching of single-cells. Lab Chip13, 3728–3734 (2013). ArticleCASGoogle Scholar
- M.Y. Hwang, S.G. Kim, H.S. Lee, and S.J. Muller: Elastic particle deformation in rectangular channel flow as a measure of particle stiffness. Soft Matter14, 216 (2017). doi: 10.1039/C7SM01829K. ArticleGoogle Scholar
- P.-H. Wu, C.M. Hale, W.-C. Chen, J.S.H. Lee, Y. Tseng, and D. Wirtz: High-throughput ballistic injection nanorheology to measure cell mechanics. Nat. Protoc.7, 155 (2012). ArticleCASGoogle Scholar
- T. Liu, X. Liu, D.R. Spring, X. Qian, J. Cui, and Z. Xu: Quantitatively mapping cellular viscosity with detailed organelle information via a designed PET fluorescent probe. Sci. Rep.4, 5418 (2014). ArticleCASGoogle Scholar
- X. Ding, Z. Peng, S.-C. S. Lin, M. Geri, S. Li, P. Li, Y. Chen, M. Dao, S. Suresh, and T.J. Huang: Cell separation using tilted-angle standing surface acoustic waves. Proc. Natl. Acad. Sci. USA111, 12992–12997 (2014). ArticleCASGoogle Scholar
- M. Islam, H. Brink, S. Blanche, C. DiPrete, T. Bongiorno, N. Stone, A. Liu, A. Philip, G. Wang, W. Lam, A. Alexeev, E.K. Waller, and T. Sulchek: Microfluidic sorting of cells by viability based on differences in cell stiffness. Sci. Rep.7, 1997 (2017). ArticleGoogle Scholar
- N.K. Gill, C. Ly, K.D. Nyberg, L. Lee, D. Qi, B. Tofig, M. Reis-Sobreiro, O. Dorigo, J. Rao, R. Wiedemeyer, B. Karlan, K. Lawrenson, M.R. Freeman, R. Damoiseaux, and A.C. Rowat: A scalable filtration method for high throughput screening based on cell deformability. Lab Chip19, 343–357 (2019). ArticleCASGoogle Scholar
- M.D. Vahey and J. Voldman: High-throughput cell and particle characterization using ISO-dielectric separation. Anal. Chem.81, 2446–2455 (2009). ArticleCASGoogle Scholar
- M.D. Vahey, L.Q. Pesudo, J.P. Svensson, L.D. Samson, and J. Voldman: Microfluidic genome-wide profiling of intrinsic electrical properties in Saccharomyces cerevisiae. Lab Chip13, 2754–2763 (2013). ArticleCASGoogle Scholar
- A. Tay, C. Murray, and D. Di Carlo: Phenotypic selection of Magnetospirillum magneticum (AMB-1) overproducers using magnetic ratcheting. Adv. Funct. Mater.27, 1703106 (2017). ArticleGoogle Scholar
- H.B. Fraser, A.E. Hirsh, L.M. Steinmetz, C. Scharfe, and M.W. Feldman: Evolutionary rate in the protein interaction network. Science296, 750–752 (2002). ArticleCASGoogle Scholar
- D.A. Drummond, J.D. Bloom, C. Adami, C.O. Wilke, and F.H. Arnold: Why highly expressed proteins evolve slowly. Proc. Natl. Acad. Sci. USA102, 14338–14343 (2005). ArticleCASGoogle Scholar
- P.Q. Nguyen. Synthetic biology engineering of biofilms as nanomaterials factories. Biochem. Soc. Trans.45, 585–597 (2017). ArticleCASGoogle Scholar
- J. Shin and V. Noireaux: An E. coli cell-free expression toolbox: application to synthetic gene circuits and artificial cells. ACS Synth. Biol.1, 29–41 (2012). ArticleCASGoogle Scholar
- Y. Schaerli and F. Hollfelder: The potential of microfluidic water-in-oil droplets in experimental biology. Mol. Biosyst.5, 1392–1404 (2009). ArticleCASGoogle Scholar
- A.J. Mach, J.H. Kim, A. Arshi, S.C. Hur, and D.D. Carlo: Automated cellular sample preparation using a centrifuge-on-a-chip. Lab Chip11, 2827–2834 (2011). ArticleCASGoogle Scholar
- N. Cheney, R. MacCurdy, J. Clune, and H. Lipson: Unshackling Evolution: Evolving Soft Robots with Multiple Materials and a Powerful Generative Encoding. In Proceedings of the 15th Annual Conference on Genetic and Evolutionary Computation (ACM, New York, NY, USA, 2013), pp. 167–174. ChapterGoogle Scholar
- G. Mackenzie, A.N. Boa, A. Diego-Taboada, S.L. Atkin, and T. Sathyapalan: Sporopollenin, The least known yet toughest natural biopolymer. Front. Mater.2, 66 (2015). ArticleGoogle Scholar
- M. Nokelainen, H. Tu, A. Vuorela, H. Notbohm, K.I. Kivirikko, and J. Myllyharju: High-level production of human type I collagen in the yeast Pichia pastoris. Yeast18, 797–806 (2001). ArticleCASGoogle Scholar
Acknowledgments
This work was supported by NSF Grant 1410751 (Division of Materials Research), the National Institutes of Health (1R01DK11077001A1), and the Wyss Institute for Biologically Inspired Engineering. The authors would also like to thank the reviewers for their time and insightful contributions to the article.